Holistic view of lifecycle costs makes for smart investments
Posted: 21 May 2017 | Nicole Wiethoff, Sebastian Meyer | No comments yet
Railway companies should take a lifecycle approach to investments in their own railway lines and include a similarly long-term rail maintenance strategy in their considerations to ensure the safety of the rail network, protect their rail infrastructure investments in the long-term and comply with the latest environmental requirements such as reduced noise levels. For European Railway Review, Sebastian Meyer, Head of Vossloh Rail Services’ Asset and Turnout Service Unit and Nicole Wiethoff, Vossloh Fastening Systems’ Vice President of System Technology, explains that Vossloh’s lifecycle cost (LCC) simulation app illustrates cost-reduction potentials based on typical examples of railway lines and provides users with a specific simulation of costs and benefits.
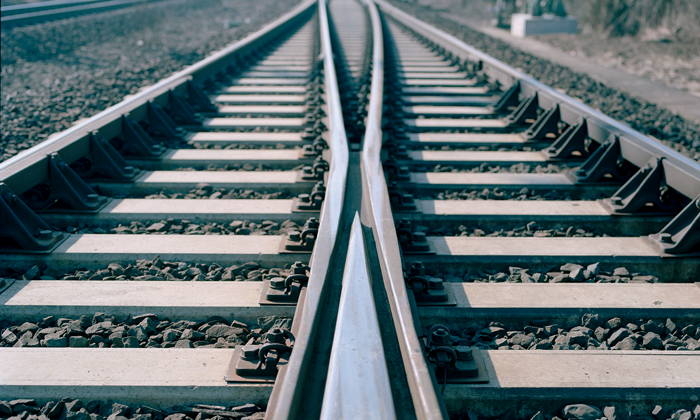
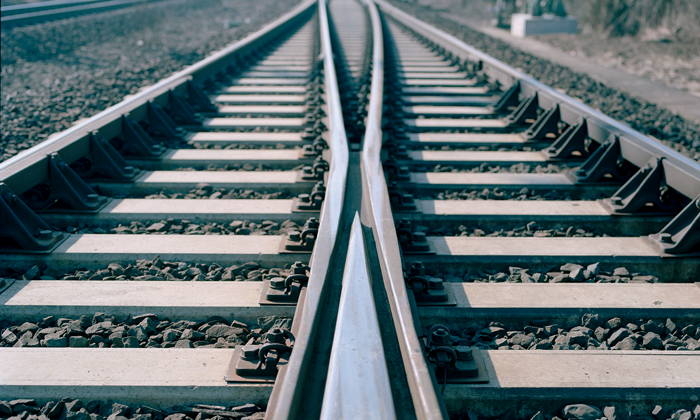
When taking their investment decision, investors and network operators should consider both the installation outlay and the subsequent maintenance intervals over the whole operating phase. After all, the selection of the infrastructure components to be installed reverberates through more than 80% of the lifecycle costs (LCC). Ideally, product design, technology and maintenance strategy go hand-in-hand.
Phases of the lifecycle
By definition, the analysis of LCC examines and evaluates the costs of a product or a system over its entire life until it is replaced or recycled. For a rail system, the lifecycle comprises four phases:
1. Planning and design
2. Realisation and installation
3. Operation and maintenance
4. Renewal and recycling.
However, the distribution of the costs over these phases varies significantly: The planning and design phase only accounts for 5% of the total costs of a railway network, while realisation and installation account for 28%, operation for 65%, and renewal and recycling for the remaining 2% (see Figure 1). These figures show that an intelligent maintenance strategy is the key to proper asset management. The right planning prevents disruptions and downtime, extends the service life of the track and ensures high operational safety standards.
Vossloh’s cost simulation illustrates how the interplay of a planning, design and maintenance strategy can be exploited to realise cost savings mainly in the operation and maintenance phase. The simulation is based on typical examples of railway lines and starts with a baseline scenario followed by a succession of optimisation steps that show which reduction in LCC can be achieved by using diverse railway infrastructure components and maintenance methods. This is then used to derive a strategy that offers the best balance of cost and benefits for the given line-specific parameters. For reasons of simplicity, the simulation is limited to the costs of material and labour and the construction-related extra costs of a simple line section without turnout systems. These are the costs that are relevant for the comparison of product alternatives in terms of both capital expenditure and maintenance costs. Since they represent only part of the total costs, they merely serve as an indication. For ease of understanding, a fair value analysis is per – formed, i.e. the impact of interest and inflation rates is not considered in the cost models. Depending on the type of the line, network operators and railway companies use different horizons for their review. Typically, a service life of approximately 40 years is assumed for ballasted track, while the horizon for the slab track of a high-speed line would be more like 60 years.
The following simulation describes the typical example of a main line1.
Main line simulation model
This model describes a typical European Union main line on ballasted track with concrete sleepers (ties) over a period of 40 years (see Figure 2). The traffic load of the 200km-long line is approximately 20 MGT per year and the maximum speed is 160km/h. Other line parameters include a radius of curvature not smaller than 3,500m and the standard rail profile 60 E1 in R260 grade.
The baseline scenario (as indicated with the black line in Figure 2) shows the development of the LCC for the ballasted track if the rail fastening system W 14 with rigid rail pad and B 70 sleepers are used. As from commissioning (t=0), regular maintenance work is required, gradually increasing the total costs over the 40 years. Therefore, in this scenario the ballast bed must be tamped every two years and the rails must be renewed every 10 years unless surface treatment is performed. Under certain circumstances, it may also be necessary to replace concrete sleepers.
In a first optimisation step of the simulation, the rail fastening system W 21 with highly elastic rail pads made of cellentic is used instead of the W 14. This system not only consists of durable and resistant materials exhibiting a significantly reduced wear, but also ensures an optimum transfer of the forces exerted by the passing trains. While the combination of the fastening system W 21 and the B 07 sleeper used in this case slightly increases the initial outlay, this is more than offset by the lower maintenance costs. Components made of cellentic demonstrably reduce vibrations and thus minimise the wear and tear of the entire permanent way. Thanks to these highly elastic components, the ballast and the ballast bed are exposed to reduced loads which results in savings on tamping and renewal costs. With otherwise unchanged parameters, the extension of the ballast tamping interval to three years alone reduces the LCC over the 40-year period by 5%.
If, in addition, the new sleeper dowel Sdü NG is used, the growth of microcracks in the concrete sleeper can be reduced as revealed in tests performed by Vossloh. The novel geometry of the dowel thread ensures a primarily perpendicular transfer of the forces into the sleeper so that lateral forces are reduced. This second optimisation step reduces the LCC of the line again by approximately 2%.
Since a replacement of the rails is costly and requires a major effort, it should always be the last resort. So in the third optimisation step of the simulation, a corrective maintenance strategy is factored in. Performed every four years, high performance milling ensures an accurate elimination of rail defects as well as a precise reprofiling of the rail. In the typical example at hand, this almost doubles the service life of the rail. Since the rails in this scenario only have to be replaced once instead of three times during period under review, the maintenance costs, the construction-related extra costs and above all the material costs are reduced substantially. According to calculations, the costs of replacing a rail in the track bed are approximately 15 times higher than the milling costs. Also, planning a rail replacement requires much more time, and hence costs, than commissioning a milling job. This optimisation step reduces the LCC by another 27%.
Mobile rail milling is one of the most advanced ways of rail treatment today. Yet in the interests of maximum use of rail infrastructure, this form of treatment is being overtaken by preventive main – tenance. A regular material removal of 0.1mm is sufficient to prevent the development of surface cracks that otherwise will spread deeper into the metal and result in rail fracture. A rail surface that is continuously kept in optimum condition reduces the wear of both the permanent way and the rolling stock.
A direct comparison of corrective and preventive maintenance of railway lines with a similar profile of use again shows that:
1. Corrective maintenance alone will not result in a complete elimination of head checks
2. Preventive maintenance right from the commissioning of the line not only offers benefits in terms of direct costs, but also contributes to a significant extension of the service life of the rail (see Figure 3).
Hence the fourth optimisation step of the simulation incorporates a preventive maintenance strategy. Given the relatively normal rail loads on conventional lines, regular high-speed grinding (HSG) prevents or minimises rail defects, corrugation, and slip waves. It optimises the longitudinal rail profile and demonstrably prevents rolling contact fatigue (head checks) and, as a spin-off, significantly lowers the noise emissions from the rail. The high working speed of up to 80km/h allows grinding without track possessions which minimises the costs resulting from construction-related extra costs (or costs of operational complications). With a tailor-made maintenance strategy comprising HSG and a single corrective milling pass after 15 years, the service life of the rail can (in this typical example) be extended by roughly 30 years. Since a smoother rail also results in less vibrations, the life expectancy of the rail pad is also substantially extended.
For a typical 200km-long line and the selected parameters, all of the aforementioned measures – from the installation of highly elastic rail fastening systems to innovative anchoring in the concrete sleeper and an intelligent maintenance strategy – together result in life-time savings of 32% (as indicated with the green line in Figure 2) over the baseline scenario, which translates into nearly €50 million.
Summary
The evaluation of the four phases of the lifecycle differs from both a time and a budget perspective. Therefore, tendering criteria should not only be focused on capital expenditures and initial costs but – especially for the cost-intensive phases of the lifecycle – more on the operation and the technical quality monitoring of the line. Such a strategy will significantly reduce the costs, extend the service life and protect the investment in the long-term. Preventive maintenance can also avoid nasty shocks and surprises while demonstrably reducing the noise emissions on the treated sections of the line.
Reference
1. Additional information and details on the LCC simulation app will be available to visitors of Vossloh’s stand at the 2017 International Exhibition for Track Technology (iaf) in Münster, Germany – Stand B-200, Outdoor Area Bahnhof B-Nord.
Biography